Volume 1, Article 12
Growth Rate Reduction of GaN Due to Ga Surface Accumulation
Devin Crawford,
Ruediger Held,
A. M. Johnston,
A. M. Dabiran,
Philip I. Cohen
Department of
Electrical Engineering, University of Minnesota
This article was received on 7/1/96 and accepted on 9/23/1996.
Abstract
GaN(0001) has been grown on Al2O3 (0001) by molecular
beam epitaxy where NH3 was used as the nitrogen precursor. Desorption
mass spectroscopy and reflection high energy electron diffraction (RHEED)
were used to monitor the relationship between growth rate and the incident
fluxes during growth. Excess surface Ga decreases the GaN formation rate
when the substrate temperature is too low or the Ga flux is too high. A
simple rate equation is used to describe the observed behavior.
1. Introduction
A number of issues related to growth kinetics of GaN must be addressed.
Historically, the n-type conductivity commonly exhibited by GaN was attributed
to N vacancies [1]
. This problem was thought to arise from a kinetic barrier to N incorporation
during growth. Recent results by Lee et. al[2]
and also by Jones et. al[3]
using NH3 as the nitrogen precursor during molecular beam epitaxy
(MBE) indicate that the GaN growth rate is strongly temperature dependent,
exhibiting a maximum between 750°C and 800°C. The high temperature
decrease in growth rate is typical of GaN, and has been attributed to Ga
desorption at elevated substrate temperature [2]
[3]
as well as to the decomposition of GaN [4]
. At lower substrate temperature the common belief is that reduced N incorporation
efficiency limits the growth rate [2]
.
In this paper we will address the low temperature issues related to
GaN MBE where the GaN decomposition rate is much less than the growth rate
. In section 3.1
we examine the transient response of the GaN surface composition and chemistry
to a step-function of Ga flux. The time dependence of the Ga desorption
after exposure of the surface to incident Ga indicates that Ga adsorbs
to the surface in two states. The first deposited layer of Ga sticks to
the surface in a strongly bound state, whereas subsequent desorption occurs
at a higher rate. The more tightly bound of these layers is observed only
when the surface has been previously exposed to NH3.
In section 3.2
we examine the relationship between growth rate, substrate temperature
and the incident fluxes. We show how desorption mass spectroscopy (DMS)
can be used to determine whether the GaN formation rate is limited by the
available N (Ga rich) or by the available Ga (N rich) and present a single
rate equation to explain a decrease in the GaN formation rate with increasing
Ga flux.
2. Experimental
Growth was carried out in a cryopumped Gen II MBE system which is shown
schematically in Figure
1. A quadrupole mass spectrometer mounted in one of the source ports
enabled detection of the type and intensity of desorbed species from the
substrate surface. The specular RHEED intensity was monitored using a photomultiplier
tube. The NH3 flux was held constant during growth using a capacitance
manometer in conjunction with a closed loop PID controller and solenoid
control valve to maintain constant pressure in the NH3 gas line
behind a manually regulated precision leak valve. A flux monitor located
on the back of the sample manipulator was used to determine the incident
beam equivalent pressure by rotating the sample manipulator. Absolute calibraiton
of the incident Ga flux is achieved by monitoring RHEED intensity oscillations
during growth of GaAs(001) in a separate experiment. Samples were prepared
by successively cleaning in acetone and methanol, which was followed by
a 5 minute etch at 70°C in 3:1 H3PO4:H2SO4.
The substrates were then rinsed in deionized water and blown dry with N2.
This process resulted in atomically smooth surfaces that exhibited
1000
Å terraces as indicated by atomic force microscopy (AFM). Without
the etch, AFM showed polish marks on the substrate surface, and no atomic
steps could be seen.
The samples were loosely mounted via mechanical support in order to
reduce thermal stress during growth. Consequently, thermal contact between
the substrate heater and the sample was reduced, and a 0.2 µm layer
of Ti was deposited on the back of the wafers to efficiently couple radiative
energy from the heater to the substrate.
3. Results
3.1. Transient Response of the Surface Composition
The transient response of the specular RHEED intensity observed along the
<011
> azimuth as
well as the response of the desorbed Ga and H2 fluxes to a step-function
of incident Ga on the GaN surface are shown in Figure
2. These data were measured after growth had been terminated and the
background NH3 pressure had been reduced to <10-9
Torr while maintaining a constant substrate temperature of 760°C. A
number of important features are observed after opening the Ga shutter.
The initially low Ga desorption flux seen in Figure
2 indicates that Ga adsorbs in a strongly bound site that is characterized
by a long residence time. We also see in Figure
2 that H2 is a byproduct of this adsorption process. After
deposition of roughly one monolayer, the Ga desorption increases and the
H2 desorption decreases, indicating the presence of a second,
weakly bound state. These results are consistent with the findings of Jones
et. al [3]
and Lee et. al[5]
who both proposed that Ga exists in two adsorption sites during growth.
After this initial Ga pulse, we close the Ga shutter and allow the excess
Ga to desorb from the surface. Subsequent exposure to incident Ga results
in only the higher Ga desorption flux, with no detectable change in the
H2 desorption, and no reduced desorption flux indicative of
the strongly bound Ga. We can again prepare a surface that will adsorb
Ga in the strongly bound sites by exposing the surface to NH3.
The procedure outlined above can be used to detect the presence of these
sites. Measurement of the RHEED intensity as shown in Figure
2 and Figure
3 reveals that two slope maxima occur during the initial transient
RHEED decrease. Quantitative information can be extracted from this RHEED
intensity variation by definition of the time interval,
t,
as shown in Figure
3. We find that the time dependence of the transient Ga and H2
desorption track this RHEED intensity variation as indicated in Figure
2. Based on this observation we believe that
t
gives an estimate of the time required for saturation of the strongly bound
Ga sites. The quantity 1/
t
decreases linearly with increasing NH3 beam equivalent pressure
(BEP), while it increases linearly with incident Ga flux. Furthermore,
this behavior is observed only when the incident Ga exceeds the available
N provided by the incident NH3.
Based on these transient data, we have identified two Ga adsorption
sites. We will present evidence in the next section that the more tightly
bound Ga site contributes to growth, whereas the weakly bound Ga acts to
inhibit growth by blocking the strongly bound Ga, and inhibiting the incorporation
of N from NH3.
3.2. Steady State Growth Behavior
RHEED indicates that high Ga flux and high substrate temperature are necessary
to achieve atomically smooth surface morphology. The objective of this
section is to examine the growth kinetics when the incident Ga exceeds
the available N provided by NH3.
In Figure
4 and Figure
5 we see the desorbed H2, N2, and Ga fluxes resulting
from exposure of the GaN surface to a 15 second pulse of Ga while the NH3
BEP is held constant. Changes in other detected species such as NHx
complexes or atomic N were too small to measure. For the data shown in
Figure
4 the Ga flux was 4.2x1014 cm-2s-1
whereas in Figure
5 it was increased to 1.4x1015 cm-2s-1 .
All other growth parameters were the same for both plots. The increased
H2 desorption during growth is attributed to the forward reaction
2 Ga + 2 NH3 -> 2 GaN + 3 H2
In the absence of incident Ga we find that contributions from the reaction
2 NH3 -> N2 + 3 H2
become significant at substrate temperatures exceeding 800°C, and we
have not yet determined the relative contributions of the two reactions
during and prior to growth at elevated temperatures. At temperatures below
800°C, however, we believe that
H2
yields a reliable estimate of the growth rate. The accuracy of this technique
is currently being investigated using post growth film thickness measurements.
In Figure
5 we see that initiation of growth causes a transient pulse of H2
to desorb from the surface, whereas in Figure
4 the desorbed H2 flux reaches its maximum value at steady-state.
In general, we find that the desorbed H2 flux during steady-state
growth increases linearly with increasing Ga flux, but then decreases as
the Ga flux exceeds a saturation value. This relationship is shown in Figure
6, where we see the dependence of
H2
on the incident Ga flux at three different substrate temperatures. In Figure
6 we see that high Ga flux causes a reduction in the growth rate, but
that increasing the substrate temperature minimizes this effect. A temperature
gradient of about 30°C exists across the substrate [6]
which introduces unertainty to the data shown in Figure
6. This uncertainty arises from the fact that DMS measurements integrate
the desorbed flux over the whole sample. This effect is particularly pronounced
at high incident Ga flux, where the growth rate is strongly temperature
dependent. This temperature gradient also makes verification of the DMS
data difficult, since film thickness measurments are dependent on the location
on the sample where the film thickness is measrued. These difficulties
can be overcome by conducting the same measurments on samples that are
known to be isothermal, and work is currently underway to achieve this
goal.
In spite of the problems introduced by the temperature gradient, we
are confident that the data in Figure
6 yield an accurate picture of the general relationship between growth
rate, fluxes, and substrate temperature. The reduced growth rate at high
Ga flux can be accounted for by consideration of the following simple kinetic
model. We start by making a number of assumptions about the atomistic behavior
of adsorbed Ga which will be justified by agreement with measured data.
First, based on the DMS measurements presented in section 3.1
we consider Ga in the weakly bound state. The fractional area of the surface
covered by weakly bound Ga is
Ga.
The second assumption is that Ga desorption occurs only from this weakly
bound state, which results in a Ga desorption term that is proportional
to
Ga.
We assume that for complete coverage (
Ga
= 1), the desorption flux is equal to the evaporation rate of Ga from liquid
Ga as developed in ref. [6]
, and therefore we approximate the Ga desorption rate as
GaF0(Tsub),
where F0(Tsub) is the desorption flux of Ga
vapor leaving liquid Ga. This assumption is consistent with the temperature
dependence of the Ga desorption data as measured using DMS. This model
does not require that Ga completely wet the GaN surface, since the shadowing
effect of droplets could account for the observed behavior as well. In
the limit of complete wetting,
Ga
is the Ga surface coverage. Direct measurement of
Ga
is complicated by the temperature gradient discussed earlier [6]
, and we have been unable to obtain a reliable measure of
Ga
due to the strong temperature dependence of the coverage.
The DMS measurements shown in Figure
6 indicate that excess Ga reduces the growth rate. We assume that NH3
reacts only with the strongly bound Ga, and that the excess Ga in the weakly
bound sites reduces the growth rate by blocking the underlying reactive
Ga sites. Further motivation for such a growth mechanism can be found in
the work of Liu and Stevenson [7]
, who found that the coexistence of Ga and GaN enhanced the decomposition
of NH3 relative to Ga alone.
We let the growth rate be proportional to the fraction of strongly bound
Ga sites that are exposed to the incident NH3, (1-
Ga).
We now consider the following quantities:
FN= total available incident N flux provided by the
NH3
FGa= total available Ga flux
GaF0(Tsub)=
Ga desorption flux where all temperature dependence will be included in
the Ga desorption term,
GaF0(Tsub).
DMS measurements of both the H2 and Ga desorption fluxes show
that the NH3 reactivity does not depend on substrate temperature
over the range (700°C-820°C), and we therefore let FN
be independent of substrate temperature. We wish to determine the steady-state
growth rate when FGa>FN. The time derivative
of the Ga coverage is given by
where the growth rate is FN(1-
Ga),
and NGa is the number of free Ga atoms on the surface.
The substrate surface area is A. Solving equation
3 for
Ga
at steady-state gives the growth rate
(The original document contained a typo in equation 4, corrected in
this copy of the paper on 01/20/98.)
The results of this model are shown as solid lines in Figure
6. As stated earlier, we estimate the desorption term F0(Tsub)
from the equilibrium vapor pressure of Ga over liquid Ga given in ref. [8]
. We see from Figure
6 that the measured data is in reasonable agreement with the steady-state
solution given in equation
4. The only parameter used to fit the predictions of equation
4 to the measured data was FN, which is equated to
the known Ga flux for which
H2
is maximum. The main points to be extracted from this discussion are that
excess Ga reduces the formation rate of GaN, and that this effect can be
minimized by increasing the substrate temperature. Additionally, we see
that a drastic reduction in growth rate occurs when FGa=F0(Tsub),
where
Ga=1
and growth is inhibited. We can also explain the increase in H2
desorption observed after closing the Ga shutter as seen in Figure
5 as follows. During growth, excess Ga resides on the surface and reduces
the growth rate by blocking the reactive sites. After closing the Ga shutter,
the excess Ga is depleted either by evaporation or by reaction with the
GaN surface. As more surface area is exposed, the growth rate increases
for a short time while the remaining surface Ga reacts with NH3
on the exposed GaN surface, resulting in the short pulse of H2
desorption from the surface.
3.3. Conclusions
Analysis of the transient H2 and Ga desorption, along with the
specular RHEED intensity show that Ga resides on the surface in both weakly
bound and a strongly bound sites. After saturation of the strongly bound
Ga sites, the Ga desorption flux increases, indicating the presence of
the more weakly bound Ga. The adsorption process of Ga into the strongly
bound sites produces H2 as a byproduct.
Analysis of the steady-state growth rate using DMS shows that excess
Ga reduces the growth rate. A rate equation giving the steady-state value
of the Ga coverage in the weakly bound state was described. The key feature
of this model is the blocking of reactive sites by the weakly bound Ga.
The model shows that by increasing the substrate temperature, the growth
rate can be increased due to reduced coverage of this excess Ga.
Acknowledgments
This work was partially supported by the ONR(N00014-93-1-0241) and AFOSR(AF/F49620-95-1-0431).
References
[1]
S. Strite, H. Morkoc , J. Vac. Sci. Technol. B 10, 1237-1266
(1992).
[2]
N.-E. Lee, R. C. Powell, Y.-W. Kim, J. E. Greene , J. Vac. Sci. Technol.
A 13, 2293-2302 (1995).
[3]
C.R. Jones, T. Lei, R. Kaspi, K.R. Evans, unpublished (1995).
[4]
N. Newman, J. Ross, M. Rubin , Appl. Phys. Lett. 62, 1242-1244
(1993).
[5]
R. C. Powell, N.-E. Lee, Y.-W. Kim, J. E. Greene , J. Appl. Phys. 73,
189-204 (1993).
[6] R.
Held, D. Crawford, A. M. Johnston, A. M. Dabiran, and P. I. Cohen, Journal
of Electronic Materials 26, 272-280 (1997)
[7]
S. S. Liu, D. A. Stevenson, J. Electrochem.Soc. 125, 1161
(1978).
[8]CRC Handbook of Chemistry and
Physics, 72nd Edition. p. 5-69 Editor: D. R. Lide (1991-1992)
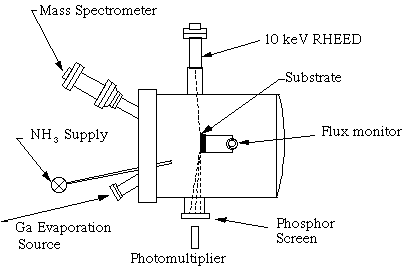 |
Figure 1. Schematic diagram
of the Gen II MBE system used for growth. |
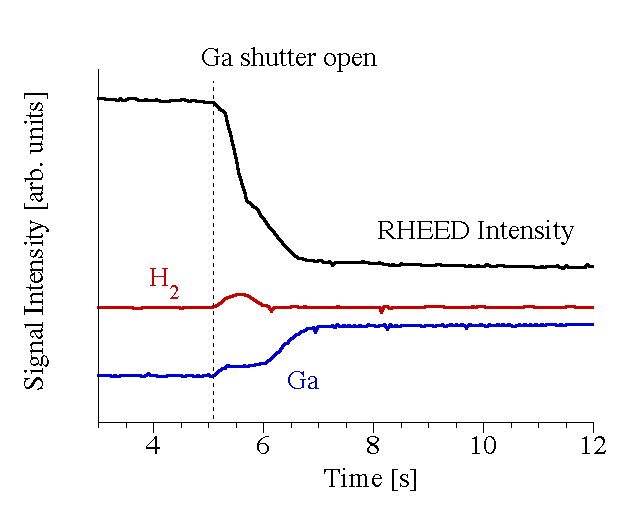 |
Figure 2. The transient response
of the Ga and H2 desorption to a step-function of incident Ga
is shown in the absence of incident NH3. The response of the
specular RHEED intensity observed along the <011 >
azimuth is also shown. (FGa=1.6x1015 cm-2s-1,
Tsub=760°C). |
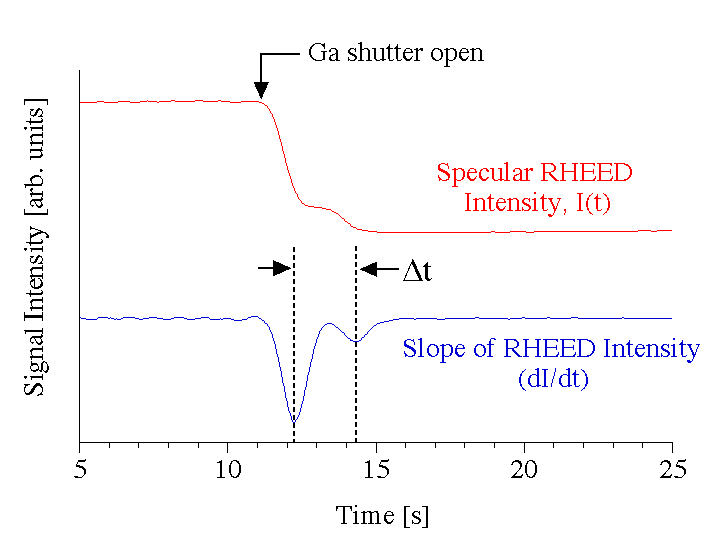 |
Figure 3. Specular RHEED intensity
and its first derivative. Differentiation of the signal allows quantitative
analysis of the transient signal. Experimental conditions for the data
shown here are Tsub=780°C, NH3 BEP=1.1x10-5
Torr. FGa = 1.45ML/s. |
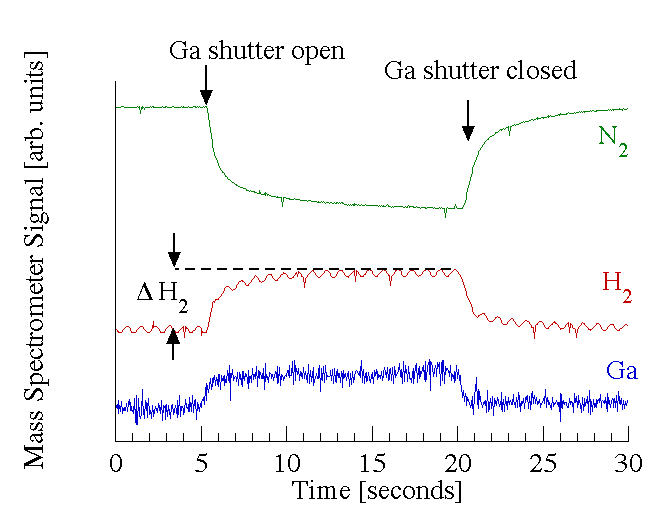 |
Figure 4. Changes in the N2,
H2 and Ga desorption are caused by exposing a smooth GaN sample
to a 15 second pulse of incident Ga under N rich growth conditions. Substrate
temperature=820°C, NH3 beam equivalent pressure = 7x10-6
Torr, Ga flux =4.2x1014 cm-2s-1. The high
frequency H2 signal oscillations arise from fluctuations in
H2 background pressure caused by temperature cycling of the
cryopumps. |
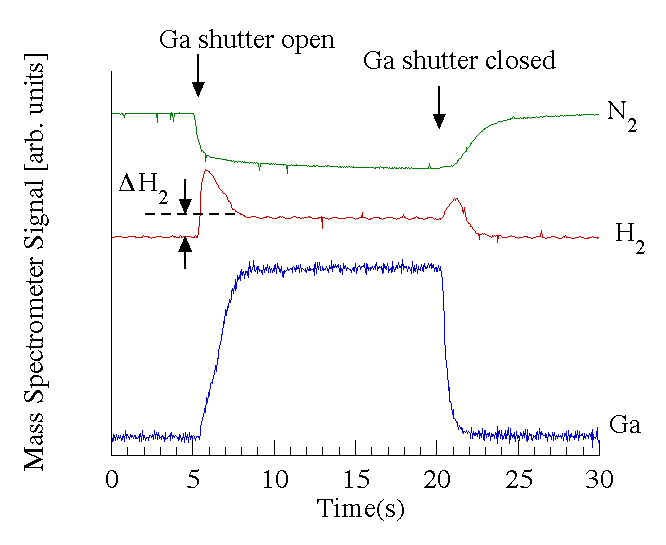 |
Figure 5. Changes in the N2,
H2 and Ga desorption are caused by exposing a smooth GaN sample
to a 15 second pulse of incident Ga under Ga rich growth conditions. Substrate
temperature=820°C, NH3 beam equivalent pressure = 7x10-6
Torr, Ga flux =1.4x1015 cm-2s-1. The H2
signal oscillations arise from fluctuations in H2 background pressure caused
by temperature cycling of the cryopumps. |
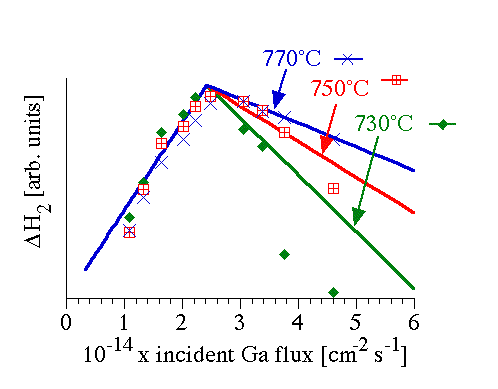 |
Figure 6. Dependence of H2
on the incident Ga flux. The lines show the theoretical incorporation rate
based on the simple kinetic model presented in this paper. |
© 1996 The Materials Research Society
Back to my home page